没有合适的资源?快使用搜索试试~ 我知道了~
温馨提示
Slow and fast light in quantum-well (QW) and quantum-dot (QD) semiconductor optical amplifiers (SOAs) using nonlinear quantum optical effects are presented. We demonstrate electrical and optical controls of fast light using the coherent population oscillation (CPO) and four wave mixing (FWM) in the gain regime of QW SOAs. We then consider the dependence on the wavelength and modal gain of the pump in QW SOAs. To enhance the tunable photonic delay of a single QW SOA, we explore a serial cascade o
资源推荐
资源详情
资源评论
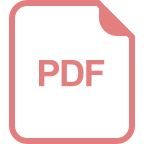
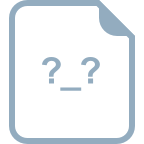
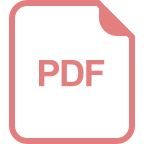
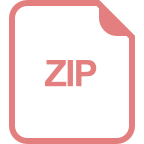
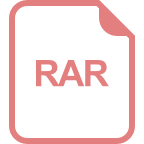
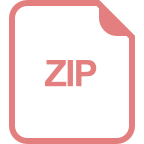
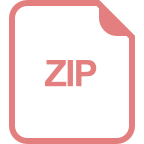
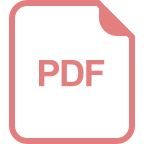
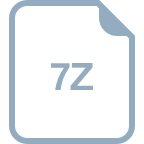
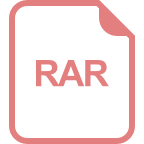
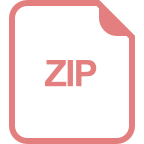
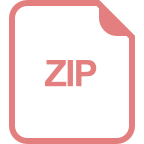
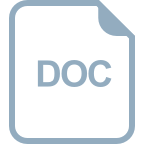
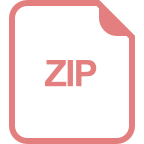
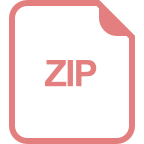
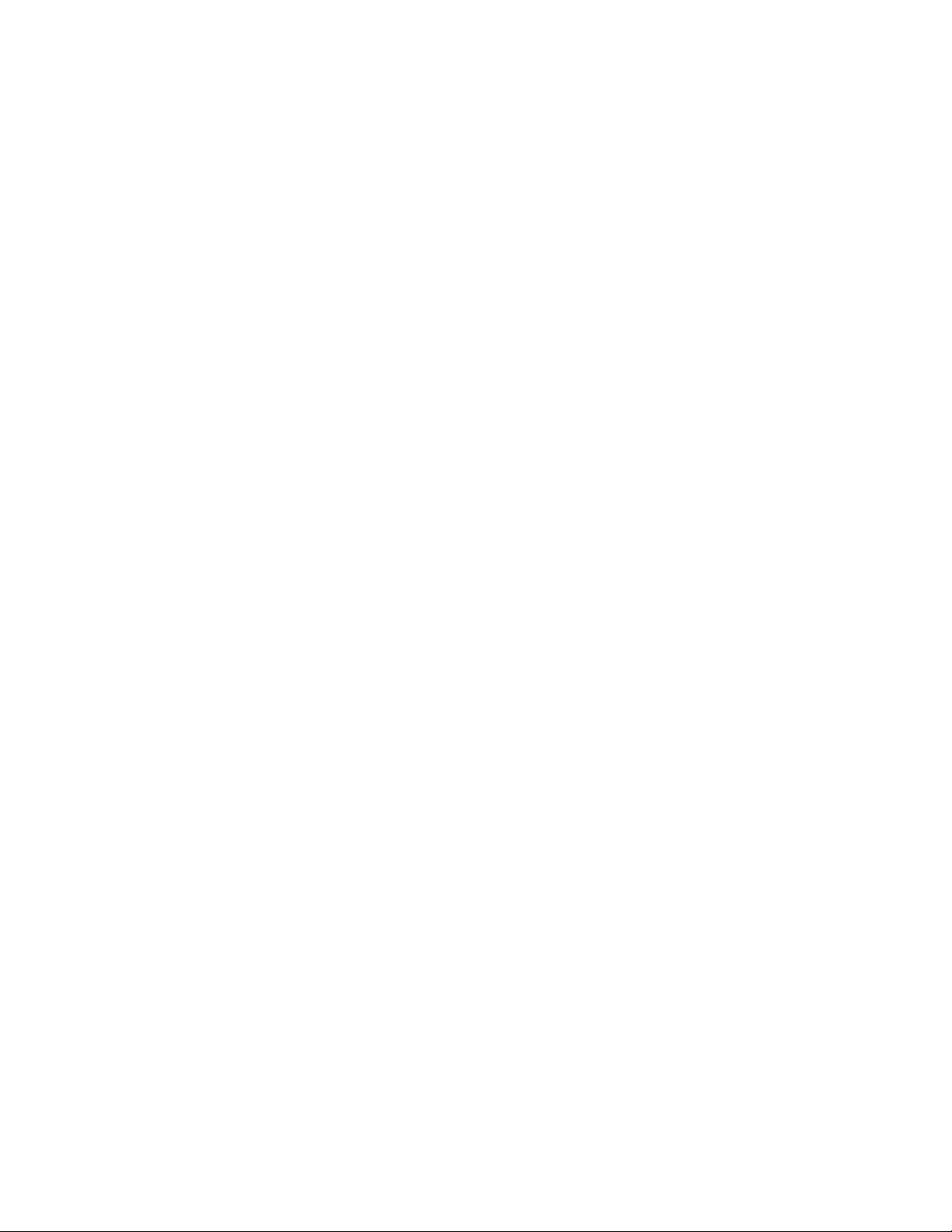
736 CHINESE OPTICS LETTERS / Vol. 6, No. 10 / October 10, 2008
Slow and fast light in quantum-well and quantum-dot
semiconductor optical amplifiers
Invited Paper
Piotr Konrad Kondratko, Akira Matsudaira, Shu-Wei Chang, and Shun Lien Chuang
Department of Electrical and Computer Engineering, University of Illinois at Urbana-Champaign, Urbana IL, 61801, USA
Received June 16, 2008
Slow and fast light in quantum-well (QW) and quantum-dot (QD) semiconductor optical amplifiers (SOAs)
using nonlinear quantum optical effects are presented. We demonstrate electrical and optical controls of
fast light using the coherent p opulation oscillation (CPO) and four wave mixing (FWM) in the gain regime
of QW SOAs. We then consider the dependence on the wavelength and modal gain of the pump in Q W
SOAs. To enhance the tunable photonic delay of a single QW SOA, we explore a serial cascade of multiple
amplifiers. A model for the number of QW SOAs in series with variable optical attenuation is developed
and matched to the experimental data. We demonstrate the scaling law and the b andwidth control by
using the serial cascade of multiple QW SOAs. Experimentally, we achieve a phase change of 160
◦
and
a scaling factor of four at 1 GHz using the cascade of four QW SOAs. Finally, we investigate CPO and
FWM slow and fast light of QD SOAs. The experiment shows that the bandwidth of the time delay as a
function of the modulation frequency changes in the absorption and gain regimes due to the carrier-lifetime
variation. The tunable phase shift in QD SOA is compared between the ground- and first excited-state
transitions with different modal gains.
OCIS codes: 230.4320, 270.1670, 250.5980.
doi: 10.3788/COL20080610.0736.
1. Introduction
A compact, electrically a nd optically tunable optical
buffer which o perates at room temperatur e is one of the
essential elements in the future optical communication
system. An all optical buffer eliminates the need for
electrical-to-optical and optical-to-electrical conversions
of the transmitted signal. It can provide data s torage to
buffer optical signals during the traffic jam of informa-
tion flow. These requirements stimulate the investigation
of the slow and fast light. Since most of the slow- and
fast-light phenomena are based on the dispersion and
phase tuning from materials or des igned spatial struc-
tures, they can also be applied to other areas such as
dispersion compensation (pulse shaping) and the o ptical
control of microwave a ntenna arrays.
There are several approaches to implement slow a nd
fast light. The conventional one is to switch the sig-
nal pulse into a fib e r loop
[1]
. This scheme can provide
high bandwidth and multi-bit delay but requires phys-
ical switching of the signal into the fiber loop. Since
the length of the fiber is fixed, the time delay is fixed.
Thus, only discrete rather than continuous tuning is pos-
sible by using different lengths of fiber s. The second
type is based on the exotic quantum or nonlinear optical
interactions between light and matter such as e le c tro-
magnetically induced transparency (EIT) and coherent
population oscillation (CPO)
[2−4]
. A large slowdown
factor is the most spectacular feature of this approach.
However, some examples in this category require ex-
treme operation conditions such as low temperature or
bulky experimental setup. Also, the bandwidth is lim-
ited by the co rresponding dynamics of the system, and in
many cases is too narrow to be used in real applications.
The third type is to utilize the filter effect from the
designed spa tial structure such as ring resonators
[5]
or
photonic-crystal waveguides
[6]
. This approach can pro-
vide the necessary tunability and bandwidth engineering.
However, the shortcoming from dispersion still requires
improvement.
For c ompact integration with active optoelectronic de-
vices, it is desirable to implement optical buffers on
semiconductors, which can als o provide electrical and
optical control of slow and fast light. The basic working
principle of slow or fast light is to manipulate the group
velocity of the wave packet by the engineering of linear
dispersion or nonlinear propagation. An example for the
first category which is commonly used in semiconduc-
tors is CPO, and an instance of the second type is the
four wave mixing (FWM), which is usually a ccompanied
with the presence of CPO. Compared with other control
schemes, semiconductor slow-light devices can offer not
only optica l
[7,8]
but also electrical control based on the
forward current injection or reverse voltage bias
[9−16]
.
This featur e makes the semiconductor unique among
other slow- a nd fa st-light schemes.
In this letter, we first discuss the fas t light based on
CPO and FWM in quantum wells (QWs). The exper-
iments of electrical and optical control of the fast-lig ht
system are then presented with new data. Wavelength
and modal gain dependences of the pump laser are ob-
served and modeled. We show that our theoretical re-
sults agree very well with experiments. Furthermore,
we demonstrate the scaling law by using casc aded QW
semiconductor optical amplifiers (SOAs) operating above
transparency. Finally, slow and fast light in quantum-dot
(QD) SOAs are investigated using absorption and gain
by changing the bias current.
2. Slow and fast light via CPO and FWM
Coherent population osc illation is the bea ting of the
carrier density induced by an intense pump and pro be
signal. Usually, the pump is a continuous-wave (CW)
1671-7694/2008/100736-07
c
2008 Chinese Optics Letters
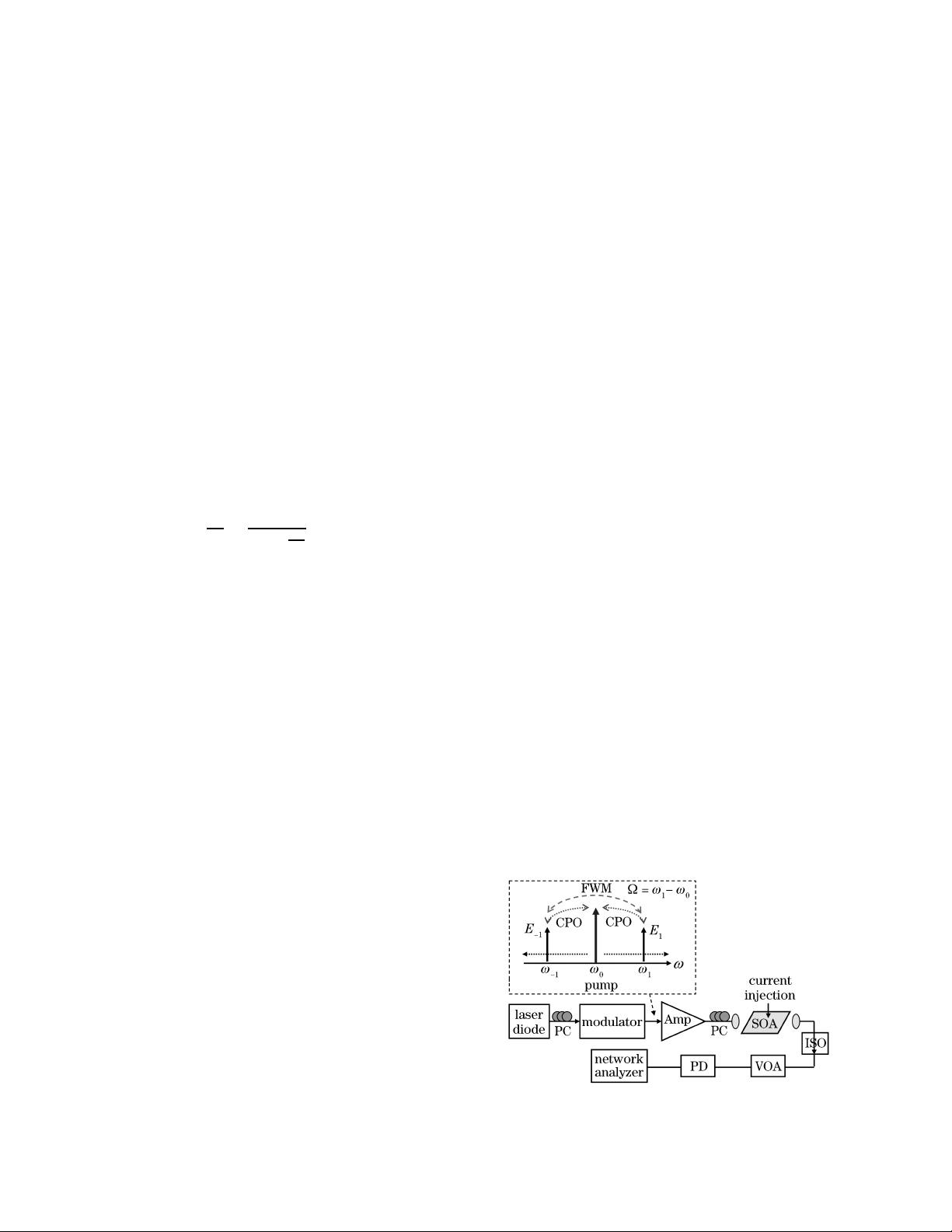
October 10, 2008 / Vol. 6, No. 10 / CHINESE OPTICS LETTERS 737
laser beam with a frequency close to the transition en-
ergies of the upper and lower states. In semiconductor
nanostructures, such states can be the first conduction
and heavy-hole (HH) valence subbands in QWs, or the
ground conduction and valence states of QDs. The in-
tense pump light saturates the abso rption or gain of the
material, depending on whether the device is biased in
the absorption or gain re gime. Suppose that the sig-
nal with a frequency detuning from the pump frequency
is incident into the material. If the detuning is much
larger than the inverse of the population lifetime, the
induced population beating cannot follow the high-speed
modulation and will have a small oscillation magnitude.
In this case, the signal simply experiences the saturated
gain and absorption. On the other hand, if the frequency
detuning is small, the magnitude of the induced beating
can be significant, and par t of the sig nal energy can be
converted into the population beating without being dis-
sipated or amplified by the medium. Corres po ndingly,
there will be an absorption or ga in dips centered at the
pump frequency on the absorption/gain spectrum. From
the Kramers Kronig relation, a positive-slop e (negative-
slope) variation of the refractive-index spectra will ac-
company this absorption (gain) dip. The corresponding
group velocity is inversely pr oportional to the group
index n
g
, which is related to this slope:
v
g
=
c
n
g
=
c
n + ω
∂n
∂ω
, (1)
where c is the vacuum speed of the light, n is the real
part of the refractive index. In the absorption regime,
the induced slope ∂n/∂ω is pos itive (a larger group index
n
g
), and thus slow light is obtained. In co ntrast, the neg-
ative slope in the gain regime (a smaller n
g
) leads to fast
light. The above picture des crib e s the linear respons e
exp erienced by the signal. In addition, the induced pop-
ulation beating can also generate a conjugate signal via
the nonlinear FWM. The effect of FWM is important in
the gain regime and cannot be discarded.
For CPO and FWM based on s e miconductor mater i-
als, there are two main effects which can influence the
slow and fast light when the electrica l curre nt injection
or reverse voltage bias is applied to the semiconductor
nanostructure. One is the change of the electronic-state
occupation, which affects the background gain or absorp-
tion. The other is the bias-dependent carrier dynamics,
which changes the linear and nonlinear susceptibilities ex-
perienced by the signal. The former determines whether
the signal experiences slowdown or speedup w hile both
factors play a significa nt role on the amount of time de-
lay, pulse broadening, and distortion.
Let us take a QD SOA as an example. Slow light is
present in the reverse-bias regime and the small forward-
bias r e gime before the zero modal gain is reached. Fur-
thermore, the recombination lifetimes (carrier dynamics)
in the forward and reverse bias regimes are different
because in the reverse-bias regime, the carriers can be
swept out of QDs by the applied electric field and thus
lead to a shorter lifetime
[11,13]
. The shorter lifetime re-
sults in a broader bandwidth for the signal, but also less
amount of time delay. O n the other hand, the lifetime
is pretty constant in the forward-bias regime before the
transparency is reached, mainly due to a constant radia-
tive lifetime. This indicates that different mechanisms
corresponding to distinct carrier dy namics may be in-
volved in the slow-light phenomena.
The even higher current injection into QDs above the
transparency level brings the slow-light regime into the
fast-light regime
[15]
as a result of the gain dip rather than
absorption dip. However, in the gain regime, in addition
to CPO, FWM also becomes important because both of
the signal and generated conjugate can be amplified and
converted to each other in the SOA. In general, for a
sinusoidally-modulated signal, the phase shift, which is
related to the amount of time advance in the fast-light
regime, is influenced by CPO and FWM simultaneously,
and their effects cannot be separated. In the gain regime,
other nonradiative recombination mechanisms also come
into play, which le ad to a shorter carrier lifetime
[15]
. The
faster carrier dynamics will again influence the a mount
of advance in the fast-light regime.
The slow and fast light in other systems such as QW or
bulk SOAs follow similar mechanis ms , but their different
carrier dynamics can show distinct characteristics of slow
and fast light. One of the key factors is the linewidth en-
hancement factor
[17]
. The linewidth enhancement factor
can lead to skewed lineshape of the gain dip, a nd as a
result, both slow and fast light can be obser ved based on
CPO in the gain regime
[18]
. Note that the effect of the
linewidth enhancement factor is not always present in the
exp eriment. For example, in the phase-shift experiment
of the sinusoida lly -modulated signal, the phase advance
of the modulated signal does not reflect the effect of the
linewidth enhancement factor
[19]
.
3. Slow and fast light experiment
Figure 1 shows the experimental setup used in the mea-
surements of CPO a nd FWM slow and fast light in the
SOA. A strong pump beam from the distributed-feedback
(DFB) laser diode is modulated by an external modulator
with a continuously variable radio frequency (RF) signa l
from a network analyzer. A modulated signal is coupled
into the SOA by fiber lense s. The magnitude and the
phase differences of RF modulated signal are measured
by the network analy z e r at different current injections
into the SOA. An erbium-doped fiber amplifier (Amp)
is inserted to preamplify the modulated input signal so
that the signal in the a bsorption regime ca n be mea-
sured. The gain of this Amp is kept constant thro ughout
the exper iment. The experiment is performed at room
Fig. 1. Experimental setup used in measuring single modu-
lated slow and fast light. VOA: variable optical attenuator;
PD: photodetector; PC: polarization controller; ISO: isolator.
剩余6页未读,继续阅读
资源评论
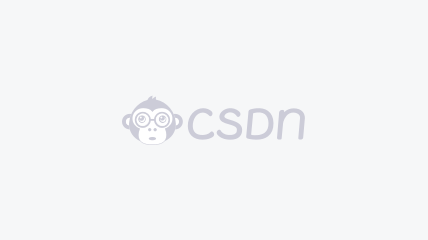

weixin_38713306
- 粉丝: 3
- 资源: 883
上传资源 快速赚钱
我的内容管理 展开
我的资源 快来上传第一个资源
我的收益
登录查看自己的收益我的积分 登录查看自己的积分
我的C币 登录后查看C币余额
我的收藏
我的下载
下载帮助

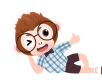
最新资源
- 电气数据417节点配电网数据
- 蒙特卡洛法场景生成+K-means聚类并削减 风电、光伏、负荷 Matlab 通过概率模型并根据weibull、beta、正态分布生成500次风电光伏、负荷场景,此基础上,基于Kmeans算法,分别对
- sgan.py 源文件,可以自行修改内容
- COVID-19 胸部 X 光图像和肺口罩图像语义分割数据
- python - 时间、日期知识汇总
- NC Cloud-集成-数据报表开发
- 基于多时间尺度滚动优化的多能源微网双层调度模型 参考文档:Collaborative Autonomous Optimization of Interconnected Multi-Energy S
- 2023-04-06-项目笔记 - 第三百七十三阶段 - 4.4.2.371全局变量的作用域-371 -2025.01.09
- python - 基础知识汇总
- 电气数据1080节点配电网数据
- 基于eNSP的企业网络规划与设计研究报告
- 2023-04-06-项目笔记 - 第三百七十三阶段 - 4.4.2.371全局变量的作用域-371 -2025.01.09
- NC Cloud-集成-业务插件注册
- VMD-LSSVM,基于VMD分解的LSSVM最小二乘支持向量机做短期电力负荷预测,预测精度非常高 结果分析 均方根误差(RMSE):0.42123 平均绝对误差(MAE):0.25901 平均相对
- 基于Python与Web前端的新年快乐动态礼花实现:代码教程和技术解析
- VMD-SSA-LSSVM,基于VMD分解的SSA优化LSSVM做短期电力负荷预测,预测精度非常高 结果分析 均方根误差(RMSE):0.17332 平均绝对误差(MAE):0.12619 平均相对
资源上传下载、课程学习等过程中有任何疑问或建议,欢迎提出宝贵意见哦~我们会及时处理!
点击此处反馈


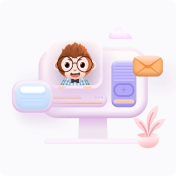
安全验证
文档复制为VIP权益,开通VIP直接复制
