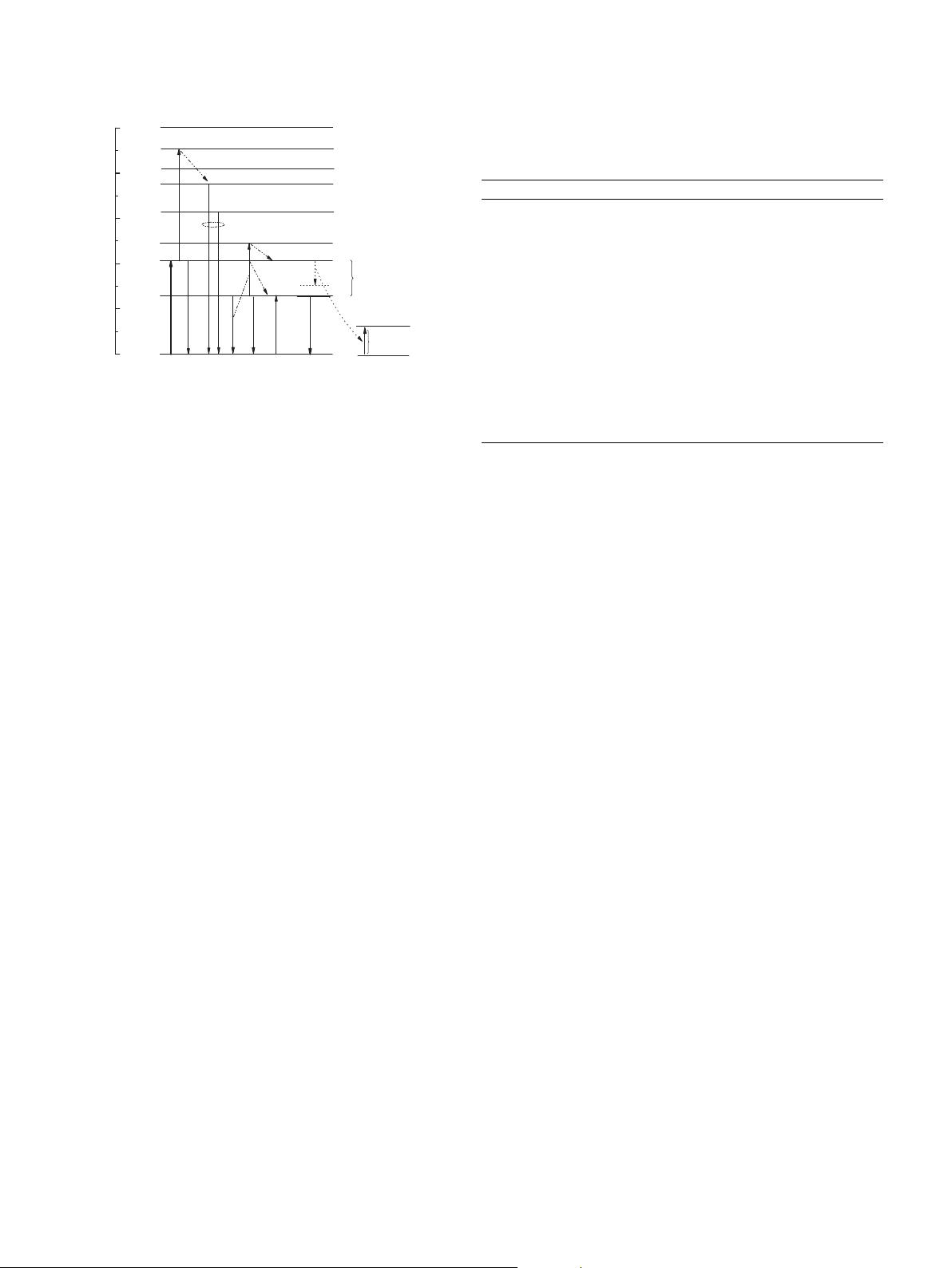
2
F
7/2
and
2
F
5/2
levels for Ce
3+
ions is about 2200 cm
1
while that for
Er
3+
ions between
4
I
11/2
and
4
I
13/2
levels is about 3600 cm
1
[15,16], which is depicted in Fig. 1, indicating that one or more
phonons of glass host will be needed to compensate this energy
difference in the realization of above ET process. Obviously, the
glass host with large phonon energy may be more beneficial.
It is known that the structural units of B
2
O
3
oxide have a rela-
tively larger phonon energy (1400 cm
1
) [17] than that of TeO
2
counterpart (750 cm
1
), which is more suitable to bridge the
energy difference between the Er
3+
:
4
I
11/2
?
4
I
13/2
transition and
the Ce
3+
:
2
F
5/2
?
2
F
7/2
transition. Meanwhile, B
2
O
3
oxide is a good
glass former, and adding B
2
O
3
oxide into glass host can enhance
its thermal stability and in turn improve the resistance for crystal-
lization [18]. In this paper, Ce
3+
ions and B
2
O
3
oxide were intro-
duced into the Er
3+
single-doped tellurite glass with composition
TeO
2
–GeO
2
–Li
2
O–Nb
2
O
5
to improve the 1.53
l
m fluorescence
emission of Er
3+
, with the aim to make full use of the ET from
Er
3+
:
4
I
11/2
level to Ce
3+
:
2
F
7/2
level meanwhile to provide a suitable
phonon energy for this ET process. The absorption spectra, fluores-
cence spectra, up-conversion emissions, Raman spectra and DSC
curves were measured to evaluate the effects of Ce
3+
ions and
B
2
O
3
oxide introductions on the 1.53
l
m band fluorescence emis-
sion, structural nature and thermal stability of glass hosts.
Meanwhile, the relevant microscopic parameters characterizing
ET between Er
3+
and Ce
3+
ions were calculated, and the 1.53
l
m
band signal gain of Er
3+
/Ce
3+
co-doped tellurite glass fiber with
B
2
O
3
oxide was studied theoretically to reveal its feasibility as a
gain medium for high gain EDFA and laser.
2. Experimental procedures
2.1. Glass preparation
The Er
3+
/Ce
3+
co-doped tellurite glasses were prepared using
conventional melt-quenching technique with molar compositions
(73 x)TeO
2
–15GeO
2
–5Li
2
O–5Nb
2
O
5
–1Er
2
O
3
–1Ce
2
O
3
–xB
2
O
3
(x =0,
3, 6 mol%), and they were labeled as TGB0, TGB1 and TGB2 corre-
sponding to B
2
O
3
amount for clarity, in which Li
2
O, B
2
O
3
compo-
nents and Er
3+
,Ce
3+
ions were introduced in Li
2
CO
3
,H
3
BO
3
,Er
2
O
3
and Ce
2
O
3
compounds, respectively. The starting materials TeO
2
,
GeO
2
,Li
2
CO
3
,Nb
2
O
5
and H
3
BO
3
were reagent-grade, Er
2
O
3
and
Ce
2
O
3
were high purity (99.99%). Batches of 10 g powders were
weighed and mixed thoroughly in a high purity crucible and
melted at temperature of about 900 °C for 0.5 h in the dry gas
atmosphere. The obtained high-temperature glass melt was casted
immediately into a preheated stainless steel mold and then
annealed at 10 °C below the glass transition temperature for 2 h
and finally cooled down to the room temperature slowly. All the
glass samples were cut and polished into the same size of
10 10 2mm
3
to meet the requirements for further spectro-
scopic measurements. The Er
3+
single-doped glass sample
(74TeO
2
–15GeO
2
–5Li
2
O–5Nb
2
O
5
–1Er
2
O
3
) was also prepared as a
reference and denoted as TGE.
2.2. Sample measurements
The glass sample density was measured based on the
Archimede principle using distilled water as an immersion liquid.
The refractive index was measured based on the minimum devia-
tion method using a prism coupler (SPA-4000) with error of 0.001.
The doped RE concentration was calculated from the measured
sample density and initial composition, and the obtained results
were listed in Table 1. The UV–Vis–NIR absorption spectrum of
glass sample was recorded using a Perkin–Elmer–Lambda 950
spectrophotometer with resolution of 1 nm, and the mid-infrared
absorption spectrum was measured using a Thermo-
Nicolet-Nexus 470 FT-IR spectrophotometer with resolution of
1cm
1
. The fluorescence emission spectrum was measured using
a Jobin Yvon Triax 550 spectrophotometer with resolution of
1 nm upon excitation of a 975 nm LD. The Raman spectrum was
measured using a confocal Horbia–Jobin–Yvon HR800 spectrome-
ter with resolution of 0.65 cm
1
upon excitation of a 488 nm
Argon ions laser. The thermal stability of glass sample was deter-
mined using a differential scanning calorimeter (DSC) of TA
Instrument Q2000 with precision of 0.1 °C at a heating rate of
10 K/min from room temperature to 600 °C. The above measure-
ments were performed at room temperature.
3. Results and discussions
3.1. Thermal stability
The glass fiber drawing is a reheating process, and any crystal-
lization during this process will increase the optical scattering loss,
which will affect the signal transmission characteristics. Therefore,
the thermal stability is very important for glass sample and relates
its subsequent practical applications. The thermal stability is gen-
erally characterized by three temperature parameters [19]: the
0
5
10
15
20
25
2200 cm
-1
1530 nm
980 nm
4
F
5/2
ESA
2
F
7/2
Ce
3+
4
F
7/2
2
H
11/2
4
S
3/2
4
I
9/2
4
I
11/2
4
I
13/2
Energy
(10
3
cm
-1
)
Er
3+
4
F
9/2
4
I
15/2
2
F
5/2
ET
3600 cm
-1
ΔE
N
1
N
3
N
2
R
13
R
31
Upconversion
C
UP
W
32
A
21
R
12
R
21
Fig. 1. The energy level diagram of Er
3+
,Ce
3+
ions and relevant transitions pumped
at 980 nm.
Table 1
Density
q
, refractive index n, concentration N
Er
of Er
3+
, concentration N
Ce
of Ce
3+
, glass
transition temperature T
g
, onset crystallization temperature T
x
, the difference DT
(=T
x
T
g
), fluorescence lifetime s
m
, spontaneous radiative transition probability A
rad
;
radiative lifetime
s
rad
and branching radio b in TGE and TGBx (x = 0,1,2) glass samples.
Glass hosts TGE TGB0 TGB1 TGB2
q
(g/cm
3
) 4.930 4.950 4.806 4.689
n (±0.001) 2.010 2.019 2.024 2.030
N
Er
(10
20
/cm
3
) 3.806 3.782 3.712 3.666
N
Ce
(10
20
/cm
3
) 0 3.782 3.712 3.666
T
g
(±0.5 °C) 397.8 398.4 399.0 401.3
T
x
(±0.5 °C) 549.2 552.1 555.1 559.7
D
T =T
x
T
g
(±0.5 °C) 151.4 153.7 156.1 158.4
4
I
13/2
?
4
I
15/2
s
m
(ms) 3.25 3.09 2.98 2.81
4
I
13/2
?
4
I
15/2
A
rad
(s
1
) 297.73 313.82 325.81 336.10
s
rad
(ms) 3.36 3.19 3.07 2.98
4
I
11/2
?
4
I
13/2
A
rad
(s
1
) 57.50 57.26 53.74 53.69
4
I
11/2
?
4
I
15/2
A
rad
(s
1
) 280.75 279.57 282.15 281.88
4
I
11/2
?
4
I
13/2
+
4
I
15/2
A
rad
(s
1
) 338.25 336.83 335.89 335.57
s
rad
(ms) 2.96 2.97 2.98 2.98
4
I
13/2
?
4
I
15/2
b 1111
4
I
11/2
?
4
I
15/2
b 0.83 0.83 0.84 0.84
4
I
11/2
?
4
I
13/2
b 0.17 0.17 0.16 0.16
150 F. Yang et al. / Optical Materials 47 (2015) 149–156