没有合适的资源?快使用搜索试试~ 我知道了~
温馨提示
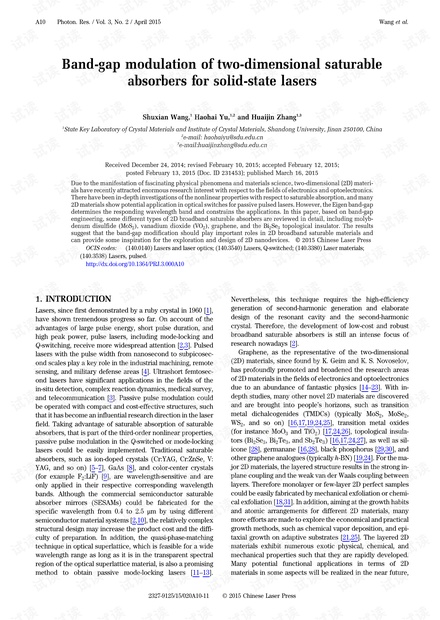

试读
11页
Due to the manifestation of fascinating physical phenomena and materials science, two-dimensional (2D) materials have recently attracted enormous research interest with respect to the fields of electronics and optoelectronics. There have been in-depth investigations of the nonlinear properties with respect to saturable absorption, and many 2D materials show potential application in optical switches for passive pulsed lasers. However, the Eigen band-gap determines the responding wavelength band a
资源推荐
资源评论
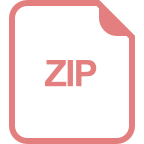
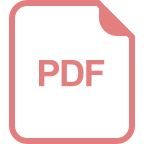
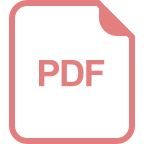
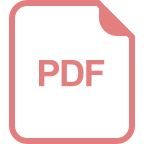
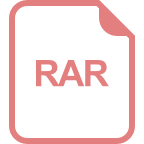
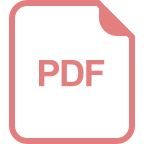
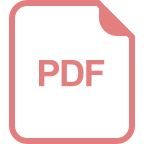
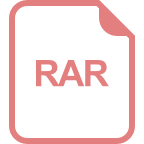
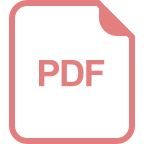
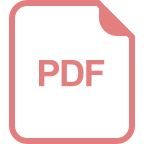
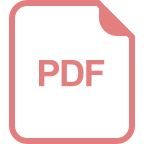
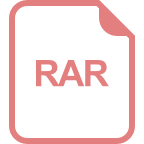
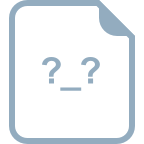
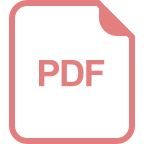
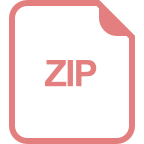
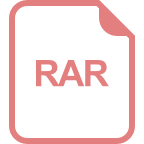
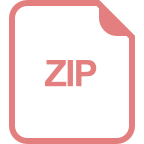
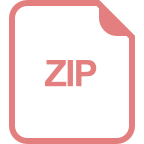
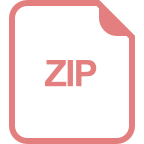
资源评论
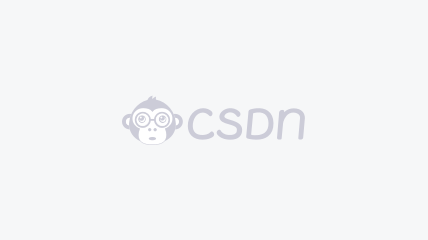

weixin_38723027
- 粉丝: 9
- 资源: 987
上传资源 快速赚钱
我的内容管理 展开
我的资源 快来上传第一个资源
我的收益
登录查看自己的收益我的积分 登录查看自己的积分
我的C币 登录后查看C币余额
我的收藏
我的下载
下载帮助

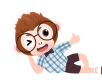
安全验证
文档复制为VIP权益,开通VIP直接复制
