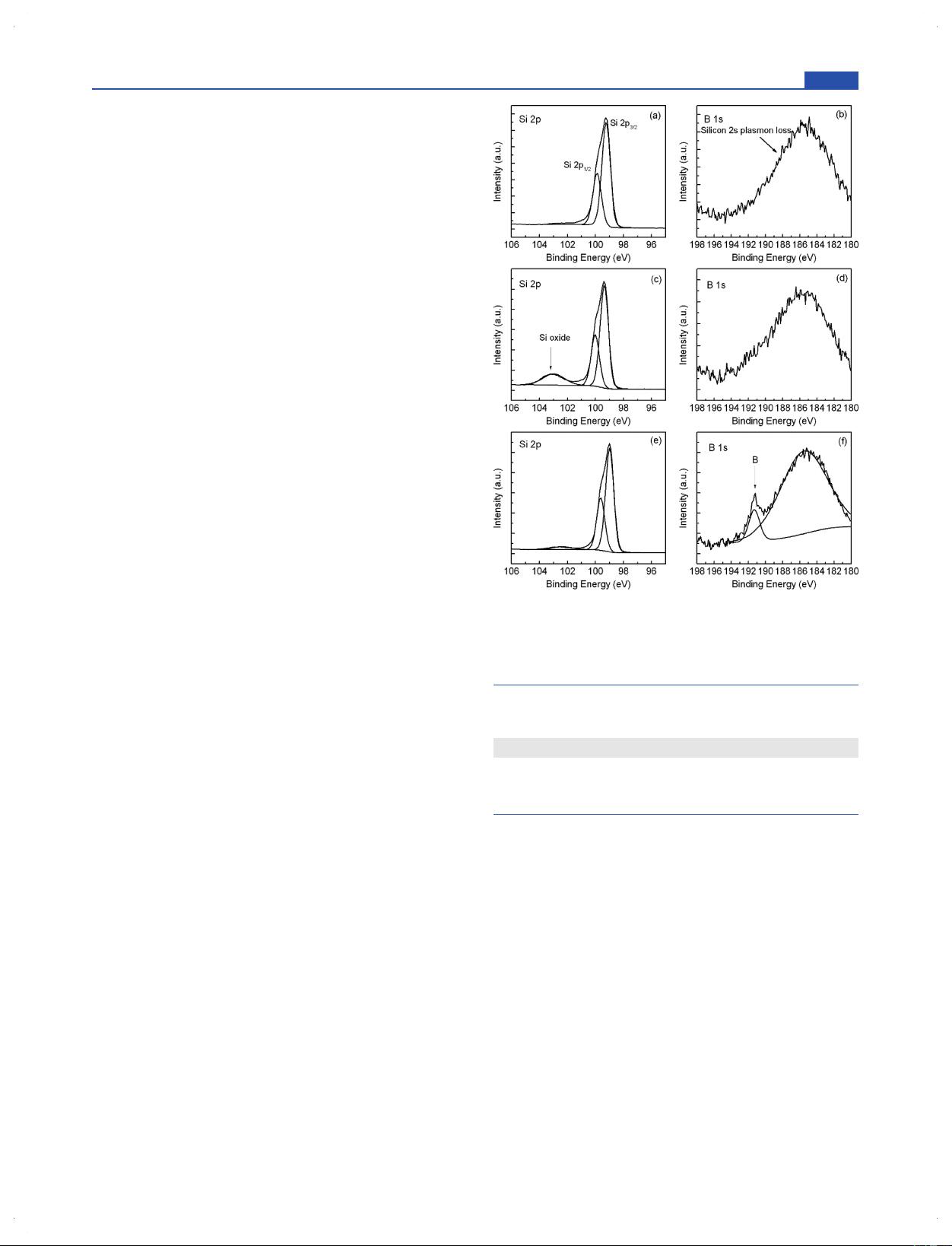
all these investigations were on phosphorus-doped silicon.
Little attention was paid to how the carbon or oxygen in
organic carriers interact with boron dopants in silicon and
whether electrically active defects exist in boron-doped silicon
via SAMM.
In this work, we investigated boron-doped silicon by SAMM
from the aspects of activation rate and defects. Allylboronic
acid pinacol ester (ABAPE) molecules were applied to
introduce boron dopants into silicon by SAMM doping. The
hole and boron dopant concentrations were measured by Hall
effect measurement and secondary ion mass spectrometry
(SIMS), respectively. To fully understand the defects in
SAMM-doped silicon, we employed deep leve l transient
spectroscopy (DLTS) and minority carrier transient spectros-
copy (MCTS) to investigate the majority and minority carrier
trap states, respectively. The results show that carbon
impurities do not bind with boron dopants. Carbon impurities
only form minority carrier trap states that have no impact on
the ionization rate of boron dopants, as a result of which boron
dopants are close to full activation. Only oxygen impurities will
bond with boron dopants, resulting in the deactivation of less
than 1% boron dopants.
■
RESULTS AND DISCUSSIONS
ABAPE monolayers were covalently bonded onto a silicon
surface following the process described in refs 1 and 15.A
compact and robust molecular monolayer was demonstrated
by co mparing X-ray photoelectron spectroscopic (XPS)
spectra of blank sample (freshly HF-etched Si), control sample
(ABAPE/p-xylene = 0:1), and ABAPE-functionalized sample
(ABAPE/p-xylene = 1:2), as shown in Figure 1 and
Supplementary Figures S2,S3.InFigure 1a, the silicon dioxide
peak (102.9 eV) is nearly invisible on the freshly HF-etched
silicon surface, and a Si 2p peak at 99.5 eV due to the Si
substrate splits into Si 2p (3/2) at 99.2 eV and Si 2p (1/2) at
99.8 eV. After heat treatment in p-xylene, the silicon dioxide
peak at 102.9 eV (fwhm 1.8 eV) shows up on the control
sample due to surface oxidation (Figure 1c). However, with a
compact ABAPE monolayer covering the silicon surface, only a
tiny silicon dioxide peak was detectable on the B-function-
alized silicon surface (Figure 1e), indicating that a compact
and robust monolayer of ABAPE has been successfully grafted.
No B 1s was detected on blank sample (Figure 1b) and control
sample (Figure 1d), but a broad peak of Si 2s plasma loss was
detected. In contrast, after functionalization with ABAPE, a
peak at 191.3 eV (fwhm 1.5 eV) was present in Figure 1f,
which is ascribed to the boron in the ABAPE monolayer. In
addition, C 1s narrow scans also support the successful
coupling of boron-containing molecules, as shown in
Supplementary Figure S3.
To evaluate the boron doping process, SAMM doping was
first performed on a high-resistivity silicon wafer (>10 kΩ cm).
Experiment details can be found in our previous publication.
15
A control sample was prepared by removing ABAPE in p-
xylene solvent. van der Pauw four-probe measurements were
applied in darkness to measure the sheet resistance. Table 1
compares the experiment results of blank sample (bare silicon
wafer), control sample, and B-doped sample. The sheet
resistance of the control sample (299.60 kΩ/□) is very
close to that of blank sample (315.82 kΩ/□), which indicates
that SAMM doping introduces negligible contamination. After
boron was doped into silicon via SAMM, the sheet resistance
dramatically decreased to 3.20 kΩ/□. This drop in sheet
resistance is exclusively attributed to molecular monolayer
doping, and hence, effective boron doping in silicon has been
demonstrated.
As we have discovered that carbon contamination from the
organic dopant carriers can deactivate as least 20% of the
phosphorus dopants in phosphorus-doped silicon by SAMM,
15
the investigations on the activation rate and defects in boron-
doped silicon became the keynote of this research. First, the
boron distribution near the silicon surface was analyzed by
SIMS. As shown in Figure 2a, the boron concentration rapidly
drops from 1.59 × 10
19
at the surface to 2.6 × 10
18
cm
−3
at ∼5
nm below the surface. The boron dopants then drop to 6.1 ×
10
15
cm
−3
at ∼104 nm below the surface. Because a monolayer
of ABAPE is grafted on the silicon surface, the total amount of
initial dopants is limited. The SIMS data from 5 to 104 nm
agrees well with the limited-source diffusion. By fitting the
limited-source diffusion model into SIMS data,
9
adiffusivity of
7.24 × 10
−15
cm
2
s
−1
was obtained, comparable to the
diffusivities for boron reported in the literature.
21
The sharp
decrease at the first 5 nm was likely related with the observed
Figure 1. XPS narrow scans of blank sample, control sample, and
ABAPE-functionalized sample. (a) and (b) are Si 2p and B 1s narrow
scans on blank sample. (c) and (d) are Si 2p and B 1s narrow scans on
control sample. (e) and (f) are Si 2p and B 1s narrow scans on
ABAPE-functionalized sample. The takeoff angle is 45°.
Table 1. Sheet Resistance of Blank Sample, Control Sample,
and Boron-Doped Samples by van der Pauw Technique
sheet resistance (kΩ/□)
blank sample 315.82
control sample 299.60
boron-doped sample 3.20
ACS Applied Electronic Materials Article
DOI: 10.1021/acsaelm.9b00748
ACS Appl. Electron. Mater. XXXX, XXX, XXX−XXX
B